Content
- Part 1: Blockchain Security
- Blockchain Basics
- How Do Blockchains Remain Secure?
- Decentralisation
- Consensus
- Settlement
- Execution
- Data Availability
- What are the Threats that Blockchains face?
- 51% Attack
- Sybil Attack
- Denial of Service (DoS)
- Eclipse Attack
- Code Exploits
- Private and Public Blockchains: What is the Difference?
- Part 2: Smart Contract Security
- Smart Contract Basics
- Common Practices for Smart Contract Security
- Testing
- Code Reviews and Audits
- Access Control and Upgradeability
- How Does Smart Contract Security Differ Between Different Applications?
- Layer 2s
- DeFi
- Governance
- Bridges
- Other
- What Are the Threats Smart Contracts Face?
- Reentrancy Vulnerabilities
- Integer Overflow and Underflow Vulnerabilities
- Logic Flaws
- Race Conditions and Transaction Ordering Attacks
- External Dependencies and Oracle Manipulation Attacks
- Other
- Part 3: The Future of Blockchain and Smart Contract Security
- Future Threats
- Quantum Computing
- The Emergence of More Advanced Hacking Techniques
- Scalability, Centralisation and Interoperability Challenges
- Other
- Part 4: A Novel Method of Securing Blockchains: Consensus Agnostic Immutability
- The Problem
- The Solution: Consensus Agnostic Immutability
- The Infrastructure
- Sharded/Modular Network
- Data Structures and Decentralised Communication
- State Storage
- Data Transmission
- P2P Communication
- Block Explorer
- Mechanism Design Safeguarding CAI
- Applications of CAI
- FAQs
- What is blockchain security?
- How does decentralization enhance blockchain security?
- What is the Byzantine Generals Problem in blockchain security?
- How does Proof of Work (PoW) contribute to blockchain security?
- What is the difference between Proof of Work (PoW) and Proof of Stake (PoS) in terms of security?
- How does blockchain settlement ensure security?
- What are the security implications of public vs. private blockchains?
- How do layer 2 solutions impact blockchain security?
- What role do nodes play in blockchain security?
- How do smart contracts factor into blockchain security?
- DISCLAIMER
12 Sep, 23
A Deep Dive Into Blockchain Security and Solutions for the Future
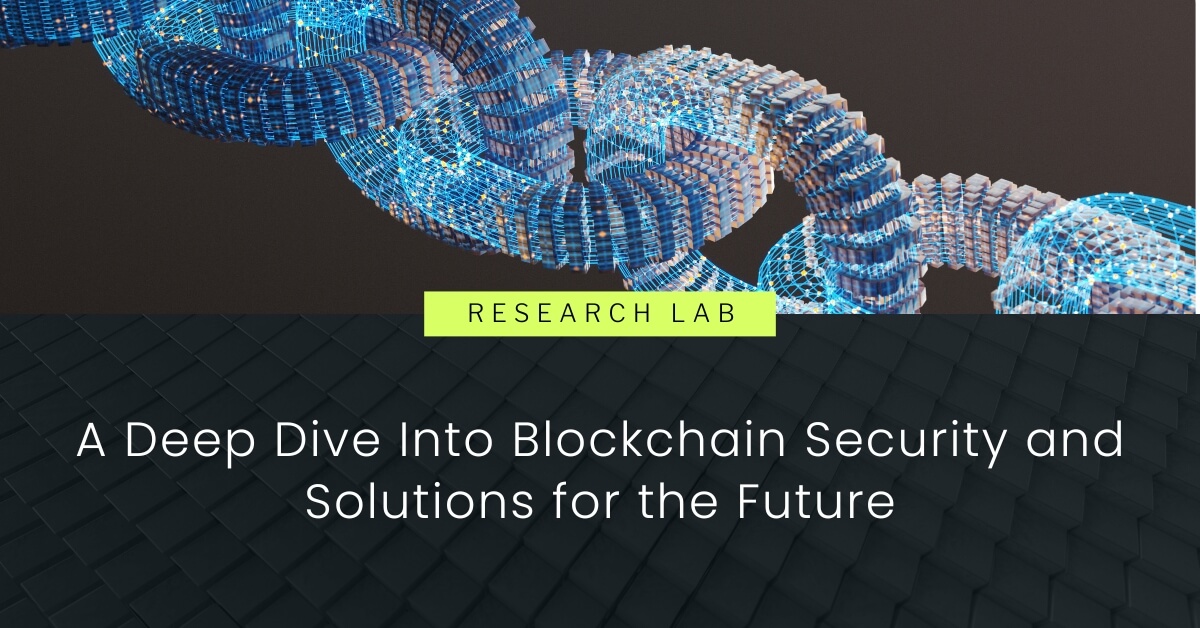
- Part 1: Blockchain Security
- Blockchain Basics
- How Do Blockchains Remain Secure?
- Decentralisation
- Consensus
- Settlement
- Execution
- Data Availability
- What are the Threats that Blockchains face?
- 51% Attack
- Sybil Attack
- Denial of Service (DoS)
- Eclipse Attack
- Code Exploits
- Private and Public Blockchains: What is the Difference?
- Part 2: Smart Contract Security
- Smart Contract Basics
- Common Practices for Smart Contract Security
- Testing
- Code Reviews and Audits
- Access Control and Upgradeability
- How Does Smart Contract Security Differ Between Different Applications?
- Layer 2s
- DeFi
- Governance
- Bridges
- Other
- What Are the Threats Smart Contracts Face?
- Reentrancy Vulnerabilities
- Integer Overflow and Underflow Vulnerabilities
- Logic Flaws
- Race Conditions and Transaction Ordering Attacks
- External Dependencies and Oracle Manipulation Attacks
- Other
- Part 3: The Future of Blockchain and Smart Contract Security
- Future Threats
- Quantum Computing
- The Emergence of More Advanced Hacking Techniques
- Scalability, Centralisation and Interoperability Challenges
- Other
- Part 4: A Novel Method of Securing Blockchains: Consensus Agnostic Immutability
- The Problem
- The Solution: Consensus Agnostic Immutability
- The Infrastructure
- Sharded/Modular Network
- Data Structures and Decentralised Communication
- State Storage
- Data Transmission
- P2P Communication
- Block Explorer
- Mechanism Design Safeguarding CAI
- Applications of CAI
- FAQs
- What is blockchain security?
- How does decentralization enhance blockchain security?
- What is the Byzantine Generals Problem in blockchain security?
- How does Proof of Work (PoW) contribute to blockchain security?
- What is the difference between Proof of Work (PoW) and Proof of Stake (PoS) in terms of security?
- How does blockchain settlement ensure security?
- What are the security implications of public vs. private blockchains?
- How do layer 2 solutions impact blockchain security?
- What role do nodes play in blockchain security?
- How do smart contracts factor into blockchain security?
- DISCLAIMER
Part 1: Blockchain Security
Blockchain networks are often thought of as a digital fortress, with indomitable security and insurmountable walls that ensure the ledger inside remains untampered. However, a more accurate conceptualisation might be an army protecting the ledger; despite its strength, the army is beset by enemies on all sides. With gaps in their lines and potential threat from without and from within, the safety of the ledger is uncertain.
Since the inception of Bitcoin and the blockchain landscape, numerous networks have fallen due to attacks and security exploits. In the face of such vulnerability, blockchains must continuously adapt and implement an array of mechanisms to ensure the continuation of their digital domains.
Blockchain Basics
At the core of blockchain technology lies a decentralised, distributed ledger that records and stores transactions in a transparent and secure manner. The significance of a completely immutable ledger maintained in a trustless manner by a decentralised network of participants cannot be understated. Prior to the blockchain, the immutability of any particular data structure was traditionally maintained by a centralised institution that users trusted to act honestly. However, this trust was often misplaced, resulting in negative externalities for the users.
Herein lies the significance of the trustless and decentralised nature of blockchain. This revolutionary system is achieved through the creation of a chain of blocks, wherein each block contains a list of validated transactions. Once appended, the blocks are cryptographically linked to their predecessors, effectively creating an immutable chain of transaction history. To maintain security and immutability, a consensus algorithm, such as Proof of Work or Proof of Stake, is employed, dictating how the network participants agree upon the validity of transactions and the creation of new blocks. With no central authority governing the network, blockchain technology enables trustless transactions and fosters a new era of peer-to-peer (P2P) collaboration, removing intermediaries and promoting a decentralised economy.
Diving deeper into the blockchain architecture, the fundamental building blocks of the system are the nodes, which are computers or servers participating in the network. Each node maintains a copy of the entire blockchain, constantly validating and relaying transaction data across the network. The nodes can be categorised into full nodes and lightweight nodes, with full nodes storing the complete blockchain history as well as executing all transaction validations, and lightweight nodes store only a subset of the blockchain and rely on full nodes for transaction validation.
Consequently, full nodes operate as the backbone of the blockchain network, preserving its security, decentralisation, and data availability. The architecture also encompasses smart contracts, and programmable scripts running on the blockchain, which automate and facilitate the execution of predefined conditions and agreements without the need for intermediaries.
In addition to the fundamental components, blockchain networks can be classified into public, private, and consortium chains, each with its distinct characteristics and use cases. Public blockchains, such as Bitcoin and Ethereum, are open to everyone, allowing any participant to engage in transactions, validate blocks, and contribute to the network’s overall security. In contrast, private blockchains restrict access to a selected group of participants, often controlled by a central authority, and are tailored to cater to the specific needs of an organisation or a group of organisations. Consortium blockchains, also known as permissioned blockchains, operate under a shared governance model where multiple entities retain control, striking a balance between the openness of public blockchains and the control of private blockchains. This hybrid model is particularly suited for use cases where data privacy, regulatory compliance, and trust among participating entities are paramount.
The rapid evolution of blockchain technology has also given birth to novel architectures and approaches that aim to address the limitations of early blockchain implementations. One such innovation is the concept of layer 2 solutions, which are built atop the base layer of existing blockchain networks, such as Ethereum, to enhance scalability, throughput, and latency. Layer 2 solutions, like rollups and state channels, process transactions off-chain and settle the results on the base layer, thereby reducing the strain on the main network and improving its overall performance. Furthermore, blockchain interoperability is being realised through networks such as Cosmos and Polkadot, which facilitate communication and asset transfer between distinct blockchain networks, enabling seamless cross-chain interactions and fostering a more interconnected, composable blockchain ecosystem.
How Do Blockchains Remain Secure?
Given that decentralisation and trustlessness are defining aspects of blockchain technology, the role of security cannot be understated. The methods via which the immutability of the ledger is maintained and the validity of the data uploaded is ensured are of paramount importance to the adoption of the technology. Therefore, blockchain security is focused on ensuring that the participants maintaining the network remain honest. Without honest nodes, the digital ledger, the data structure upon which the blockchain is built, cannot be maintained or updated in a verifiable manner, compromising the integrity of the network and causing users to lose trust. Thus, the question arises, how is the integrity of network participants ensured and how do blockchains remain secure?
Decentralisation
Decentralisation plays a pivotal role in ensuring security and incentivising the widespread adoption of blockchain technology. By distributing the control and maintenance of the network across multiple nodes, rather than a single central authority, blockchain networks inherently discourage manipulation and fraud. This aspect of decentralisation is integral to the security of the system, as it diminishes the chances of a single point of failure and significantly reduces the potential for malicious attacks. Consequently, decentralisation fosters an environment where users can trust the integrity of the network and the transactions it processes.
The history and philosophy of blockchain technology are deeply intertwined with the principles of decentralisation that developed in response to the deficits of the traditional financial system. Specifically, the 2008 Global Financial Crisis (GFC) revealed the inherent flaws within the centralised financial systems that dominate traditional finance (TradFi), as well as the perceived greed, recklessness, and lack of accountability that characterised the actions of many key players. Banks and financial institutions, once regarded as bastions of stability, were exposed for their irresponsible lending practices and excessive risk-taking. Governments and regulatory bodies were similarly criticised for their inadequate oversight and failure to prevent the crisis. In the wake of this widespread financial turmoil, countless individuals and businesses suffered from job losses, bankruptcies, and a general erosion of trust in the existing financial system.
It was against this backdrop that Satoshi Nakamoto introduced Bitcoin, a decentralised digital currency designed to provide a more secure and transparent alternative to the centralised financial system. The core principles of Bitcoin, decentralisation, censorship resistance, and trustlessness, were a direct response to the flaws revealed during the GFC. By removing the need for intermediaries and allowing individuals to transact directly with one another, Bitcoin sought to minimise the potential for manipulation, fraud, and corruption that had plagued the traditional financial system. Furthermore, Bitcoin’s underlying blockchain technology enabled a decentralised ledger system that could maintain a transparent and tamper-proof record of transactions, ensuring that no single entity could control or manipulate the flow of information. This vision laid the foundation for the development of blockchain technology and inspired the broader cryptocurrency movement.
The principles of decentralisation and individual liberty have attracted a diverse community of blockchain enthusiasts, from libertarians to Cypherpunks. These individuals share a common belief in the importance of privacy, security, and autonomy in the digital realm. The Cypherpunk movement, in particular, has been instrumental in the early development and adoption of blockchain technology, as its values align with those of decentralisation and individual autonomy. This shared ideology has not only helped shape the trajectory of the blockchain industry but has also contributed to the technology’s resilience and adaptability in the face of various challenges.
Decentralisation is not only vital for the security of blockchain networks but also essential for driving their adoption. By fostering trustless environments, decentralisation ensures that users can transact with one another without relying on intermediaries, such as banks or payment processors. This trustless nature of the technology greatly reduces transaction costs and complexities, making blockchain networks more accessible and attractive to a wide range of users. As the underlying philosophy of decentralisation continues to resonate with users, the adoption of blockchain technology is expected to grow, ultimately transforming the way individuals and businesses interact with one another and manage their financial resources.
Consensus
Consensus can be classified as the complete set of protocols and incentives put in place by blockchain networks to ensure that the array of nodes remains in agreement with regard to the state of the ledger. Thanks to consensus, blockchain nodes can act as a replicated state machine whereby each node works together to maintain an identical copy of a state and execute the same sequence of operations. Consensus mechanisms play a crucial role in ensuring the security and integrity of blockchain networks by establishing a consistent and transparent process for validating transactions and adding new blocks to the chain.
Specifically, consensus mechanisms are designed to solve the Byzantine Generals Problem, a theoretical dilemma in the field of distributed computing, which illustrates the challenges faced by a group of generals attempting to coordinate their attack plans while simultaneously dealing with the possibility of treachery among their ranks. The crux of the problem lies in the need to establish a consensus among the generals despite the potential presence of traitorous generals who might send false information or manipulate messages to disrupt the coordination.
This problem is particularly relevant to the blockchain ecosystem, as it represents a key challenge in the design and operation of decentralised networks. In a blockchain, numerous nodes distributed across the globe work together to validate and record transactions, without the need for a central authority. These nodes must reach a consensus on the state of the distributed ledger, even in the presence of malicious actors who might attempt to manipulate the system for their benefit. The consensus employed by blockchain networks, such as Proof of Work or Proof of Stake, is specifically designed to address the Byzantine Generals Problem by ensuring that a majority of the network’s participants can agree on the state of a network.
By implementing robust consensus mechanisms that address the Byzantine Generals Problem, blockchain networks can maintain a secure, decentralised, and tamper-proof record of transactions, fostering trust and transparency within the ecosystem. The pivotal role of consensus mechanisms in blockchain security cannot be overstated; it is through their robust and tamper-proof design that decentralised networks remain resistant to malicious actors and maintain their trustless nature, even in the absence of a central governing authority.
Proof of Work (PoW) is the original consensus mechanism that laid the foundation for the inception of blockchain technology, most notably with its implementation in the Bitcoin network. The concept of PoW predates cryptocurrencies and was first introduced by Cynthia Dwork and Moni Naor in 1993 as a way to combat spam emails and denial-of-service attacks. The idea behind PoW is to require a user to perform some computational work before sending a message or request, creating a small cost that would discourage spamming. When Satoshi Nakamoto developed Bitcoin, PoW was adapted as the consensus mechanism to secure the network, validate transactions, and maintain a decentralised and trustless system.
Diving deeper into the technical details of PoW, the process involves network nodes, also known as miners, competing to solve complex cryptographic puzzles that require significant computational power. In PoW systems like Bitcoin, these puzzles are based on a cryptographic hash function (specifically, SHA-256 in Bitcoin’s case). The miners attempt to find a nonce (a random number) that, when combined with the data from the candidate block and passed through the hash function, produces a hash value that meets a specific condition, such as having a certain number of leading zeros. The difficulty of this puzzle is adjusted periodically, ensuring that the rate at which new blocks are added to the blockchain remains relatively constant.
The first miner to successfully solve the puzzle broadcasts their solution to the network, which other nodes then verify. Once verified, the miner is granted the privilege of adding the new block of transactions to the blockchain. They are also rewarded with newly minted tokens (e.g., BTCs) and transaction fees paid by the users whose transactions are included in the block. The competitive and resource-intensive nature of PoW ensures that nodes remain honest, as any attempt to manipulate the blockchain would require control over more than 50% of the network’s total computing power. This makes such attacks highly improbable and economically unfeasible. However, PoW has faced criticism for its substantial energy consumption and potential for centralisation, as mining operations become increasingly concentrated among a few dominant players with access to vast computational resources.
Proof of Stake (PoS) was conceived as an alternative consensus mechanism to address the limitations and concerns associated with PoW, such as its high energy consumption and potential centralisation. The concept of PoS was first proposed by Sunny King and Scott Nadal in 2012 in their whitepaper for Peercoin, a cryptocurrency that combined both PoW and PoS mechanisms to secure its network. The primary motivation for developing PoS was to create a more energy-efficient and environmentally friendly consensus mechanism that still maintained the decentralisation and security features inherent in PoW-based systems.
PoS emerged as a novel approach, emphasising the role of economic incentives in securing the network rather than relying on computational resources. By tying the likelihood of validating and adding new blocks to the blockchain to the amount of tokens a node holds or “stakes” as collateral, PoS encourages honest behaviour among network participants. Nodes with a greater stake in the network have more to lose if the system’s integrity is compromised, making them less likely to engage in malicious activities. This incentive structure has attracted interest from various blockchain projects, leading to the widespread adoption of PoS and its variants in numerous networks.
Diving deeper into the technical details of PoS, the selection process for validators (also known as forgers or block producers) is typically based on a combination of factors, including the number of tokens staked, the age of those tokens, and a randomised selection process. Once chosen, the validator is responsible for validating transactions, adding new blocks to the chain, and receiving the associated rewards. Unlike PoW, PoS does not require validators to solve complex cryptographic puzzles, which significantly reduces energy consumption.
To further enhance security and deter potential attacks, many PoS systems incorporate additional features such as penalties for malicious behaviour. For instance, some PoS networks implement a mechanism called “slashing” to penalise validators who attempt to validate fraudulent transactions or manipulate the blockchain. Slashing usually results in the confiscation of a portion or all of the staked tokens. This slashing is usually achieved through burning tokens, or sending them to a locked address such as 0x0, thereby creating a strong deterrent against dishonest actions.
PoS has gained considerable traction in recent years due to its energy efficiency and lower susceptibility to centralization. Prominent blockchain networks like Ethereum are in the process of transitioning from PoW to PoS, as seen with the Ethereum 2.0 upgrade, in an effort to build more sustainable, scalable, and environmentally friendly platforms. As a result, PoS is increasingly seen as a viable alternative to PoW for securing blockchain networks and maintaining the core values of decentralisation and trustlessness.
Although PoS and PoW are the predominant consensus mechanisms in the present blockchain landscape, they are by no means the only mechanisms available. Delegated Proof of Stake (DPoS), for instance, is another consensus mechanism that builds upon the PoS model by introducing a layer of representative democracy to the validation process. In DPoS systems, token holders elect a fixed number of delegates, who are then responsible for validating transactions and maintaining the network’s security. By delegating the consensus process to a smaller group of nodes, DPoS aims to achieve greater scalability and efficiency compared to traditional PoS systems, while still retaining the core security features of staking and economic incentives. Delegates are held accountable for their actions through a continuous voting process, ensuring that any misbehaving or underperforming delegates can be quickly replaced by the community. DPoS has been adopted by several blockchain networks, such as EOS and Tron, as a means of providing both enhanced security and performance for their platforms.
Byzantine Fault Tolerance (BFT) is a consensus mechanism designed to address the Byzantine Generals Problem. In the context of blockchain networks, BFT algorithms ensure that nodes can reach consensus on the state of the network, even in the presence of malicious or faulty nodes. BFT based consensus mechanisms provide strong security guarantees and can typically reach consensus with lower latency and greater finality compared to PoW and PoS systems.
Practical Byzantine Fault Tolerance (pBFT) is a widely adopted BFT consensus algorithm that operates on the principle of majority voting, requiring a supermajority (typically two-thirds or more) of nodes to agree on the validity of a proposed block before it can be added to the chain. In pBFT systems, a designated primary node proposes new blocks, while other nodes validate and vote on the proposed block in a series of communication rounds. If the primary node is found to be malicious or unresponsive, it can be replaced through a view-change mechanism, ensuring that the consensus process can continue uninterrupted. pBFT-based consensus mechanisms offer robust security and rapid finality, making them well-suited for applications that demand high transaction throughput and low latency, such as enterprise blockchain solutions and financial systems. Notable examples of pBFT implementations include the Hyperledger Fabric platform and the Tendermint consensus engine used in the Cosmos network.
Other than PoW, PoS and BFT based consensus algorithms, there exists a number of novel consensus mechanisms employed by various protocols used throughout the blockchain landscape.
Settlement
Transaction settlement refers to the process of finalising, verifying, and securely adding transactions to the distributed ledger, that is an integral aspect of the overall blockchain architecture. Although they are often conflated, the process of settlement differs from consensus, which deals with the agreement of the network participants on the validity and order of transactions. While consensus mechanisms such as PoW and PoS play a crucial role in the overall functioning of a blockchain, they primarily focus on establishing agreement among the network participants. In contrast, the settlement process is concerned with ensuring the transactions are valid before finalising them into blocks.
The significance of transaction settlement in terms of security cannot be overstated, as it forms the basis for the decentralised trust model that underpins blockchain technology. By verifying the validity of executed transactions and finalising them into blocks, settlement safeguards the network from fraud and malicious activities, ensuring that the users’ assets and sensitive data remain secure. Furthermore, the assurance of transaction validity in proposed blocks serves to relegate fraud to nodes tampering with records during consensus rather than users attempting to push fraudulent transactions into blocks. Additionally, the settlement process provides users with confidence that their transactions have been successfully completed, without the possibility of reversal or double spending.
Settlement mechanisms in different blockchains may vary in terms of their design and implementation, depending on the unique requirements and goals of the respective networks. For instance, on the Bitcoin network, the settlement process occurs between transaction validation and the PoW consensus phase. When a miner selects transactions from their mempool to include in a new block, they prioritise transactions based on their fees, ensuring that the block size does not exceed the network’s limit.
Once a set of transactions is chosen for the candidate block, the miner proceeds to execute them, effectively updating the Unspent Transaction Output (UTXO) set. The UTXO set represents the state of the Bitcoin network, keeping track of all unspent transaction outputs, which serve as the basis for future transactions. Each transaction in the candidate block refers to one or more UTXOs as inputs, marking them as spent, and creates new UTXOs as outputs for the recipients. By processing the transactions in the block and updating the UTXO set, the miner establishes a new state for the Bitcoin network, with transaction outputs redistributed among the participating addresses. This state is then preserved in the candidate block, which is subjected to the PoW consensus to be validated and appended to the blockchain.
On the Ethereum network, the settlement process likewise occurs between transaction execution and the consensus process. However, in contrast Ethereum features a complex execution environment known as the Ethereum Virtual Machine (EVM), which allows for the execution of smart contracts and more versatile transactions. Upon selecting a set of transactions for a candidate block, a validator processes the transactions in the order they appear in the block.
Each transaction finalisation updates the state of the Ethereum network, including account balances, smart contract storage, and contract code. The transaction sender’s account is debited the transaction’s value and gas fees, while the recipient’s account is credited the transaction’s value. For smart contract interactions, the EVM executes the contract code, potentially updating the contract’s storage or triggering other transactions. The final state of the network, including account balances and smart contract data, is then recorded in the candidate block.
The importance of transaction settlement in blockchain networks lies in its crucial role in upholding the security, stability, and integrity of the system. By ensuring that transactions are properly validated, executed, and recorded in candidate blocks, settlement mechanisms prevent invalid or malicious transactions from being included in the blockchain. Once a transaction has been settled the state of the network is finalised unless an issue in consensus arises. This ensures that users can trust the state of the network and the robustness of their transactions.
Execution
Transaction execution is the process through which submitted transactions are processed and validated by the network nodes before being considered for inclusion in a candidate block. This is a critical component of the blockchain security model, as it ensures that only legitimate and valid transactions are considered for settlement and subsequently added to the blockchain. The transaction execution process varies among different blockchains, as it depends on the specific network architecture and transaction models employed.
In the context of security, transaction execution serves as a preliminary layer of defence against fraudulent or malicious activities. By requiring network nodes to process and validate transactions, it ensures that invalid or improperly formatted transactions are rejected and prevented from entering the settlement stage. Moreover, during transaction execution, nodes verify that the transaction sender has the necessary funds or permissions to initiate the transaction, effectively safeguarding against attempts to double-spend or unauthorised transactions. The rigorous validation and processing of transactions during the execution phase serve to maintain the decentralised trust model that underpins blockchain technology, fostering confidence in the system and its ability to securely handle users’ assets and data.
On the Bitcoin network, transaction execution is primarily concerned with validating the inputs and outputs of a transaction. When a node receives a transaction, it checks whether the transaction inputs correspond to valid UTXOs and whether the transaction adheres to the network’s rules, such as ensuring the sum of the inputs equals or exceeds the sum of the outputs. Additionally, nodes verify the transaction signatures, confirming that the transaction has been authorised by the rightful owner of the associated private keys.
In Ethereum, transaction execution, as exhibited in the graphic below, is more complex, as the network supports a wide range of transaction types and interactions with smart contracts. The EVM serves as the execution environment, processing transactions and performing computations as specified by the smart contract code. When a transaction is received by a node, it validates the transaction format, checks the sender’s account balance and nonce, and calculates the required gas fees. For transactions involving smart contract interactions, the EVM executes the contract code, updating the contract storage and potentially triggering additional transactions as specified by the contract logic.
Data Availability
Data availability is a critical aspect of blockchain security, as it pertains to the accessibility and distribution of data stored on the blockchain, ensuring that all network participants can access and verify the information at any given time. A fundamental component of the decentralised trust model, data availability is essential for maintaining the transparency, integrity, and resilience of blockchain networks. While consensus mechanisms and transaction settlement processes contribute to the overall security and reliability of a blockchain, data availability serves as the foundation upon which these processes depend, enabling the storage and accessibility of the blockchain itself.
The role of data availability in blockchain security is multifaceted, as it not only promotes transparency and accessibility but also helps to prevent certain types of attacks and vulnerabilities. By ensuring that all network participants can access the same data, data availability prevents the risk of data tampering or manipulation, as any fraudulent changes to the blockchain would be easily detectable and rejected by the network. Moreover, data availability enables nodes to independently verify the validity and authenticity of transactions and blocks, reinforcing the decentralised trust model and eliminating the need for centralised authorities or intermediaries.
In the context of data availability, it is essential to consider the various technical aspects that contribute to the reliable distribution and accessibility of data within a blockchain network. One such aspect is the replication of data across multiple nodes, ensuring redundancy and resiliency in the face of potential failures or attacks. In distributed systems, replication can be achieved through various techniques, such as full replication, where every node stores a complete copy of the data, or sharding, where nodes store only a portion of the data, relying on other nodes to access the remaining information.
Different blockchain networks, such as Bitcoin and Ethereum, employ various techniques and strategies to ensure data availability. In both networks, full nodes maintain a complete copy of the blockchain, allowing for independent verification of transaction history and providing redundancy to keep the network secure even if a significant number of nodes are compromised or go offline. Both networks use Merkle tries for efficient data representation and verification. Ethereum, with its added complexity of smart contracts and diverse data types, introduces decentralised storage solutions like Swarm and IPFS protocols for off-chain data, enhancing data availability and redundancy. These protocols use content-addressable storage, ensuring data integrity and efficient retrieval. Ethereum also employs a trie-based data structure called the Patricia Merkle trie to store its state, enabling efficient updates, verifications, and proofs of non-inclusion. Merkle Tries (which Patricia Merkle Tries are a variation of) are data structures used to encode the state of the system, composed of various transactions merged together to form a single hash referred to as the ‘root node’, as illustrated below, that stores the current state of the system.
Sharding is a technique that partitions the blockchain into smaller, more manageable pieces known as shards. Each shard is responsible for processing and storing a subset of transactions, thereby distributing the load and improving the overall scalability of the network. In sharded environments, maintaining data availability is crucial for security, as it ensures that nodes can still access and verify the necessary information, even if they do not store the entire blockchain. Various strategies, such as cross-shard communication and shard validation, are employed to maintain data availability in sharded networks, ultimately contributing to the security and integrity of the system.
Data availability sampling is another approach that allows network participants to verify the presence and accessibility of data without downloading the entire data set. By randomly sampling small parts of a shard or block, nodes can efficiently detect any inconsistencies or unavailability of data. In case of any discrepancies, the network can then take corrective action, such as penalising the malicious participant or discarding the invalid data. Data availability proofs, on the other hand, allow nodes to demonstrate that a piece of data is accessible and retrievable by the network, even if the node itself does not possess the entire data set. This technique is particularly useful in sharded environments, where nodes may not store complete copies of the blockchain.
Erasure coding is another system that can be employed to enhance data availability, particularly in sharded networks. It involves breaking data into smaller fragments and encoding them with additional redundant data, allowing the original data to be reconstructed even if some fragments are lost or unavailable. This provides an additional layer of resiliency, ensuring that data remains accessible and verifiable by the network, even in the face of failures or attacks. Erasure coding is often employed in conjunction with systems such as data availability sampling (DAS) to provide data availability guarantees in a reliable and efficient manner while also ensuring the redundancy of the data itself.
Cryptography
Cryptography mechanisms are indispensable components of blockchain security as they provide the foundation upon which trustless and secure transactions can take place in a decentralised network. In essence, cryptography is the art of secure communication, involving the application of mathematical techniques to render data unreadable by unauthorised parties. Blockchain networks like Bitcoin and Ethereum rely heavily on various cryptographic algorithms to secure transactions, verify identities, and ensure the integrity of the entire system. By offering robust privacy, authentication, and data integrity measures, these cryptographic mechanisms form the bedrock of blockchain security, enabling users to engage in trustless interactions and ensuring the resilience of the network against potential attacks.
Public-key cryptography, also known as asymmetric cryptography, is a central aspect of blockchain security. This cryptographic technique employs a pair of keys, a public key and a private key, for encryption and decryption processes. The public key is openly shared and used to encrypt data, while the private key remains secret and is used to decrypt the encrypted data. In the context of blockchain networks, public-key cryptography is employed to generate digital signatures, which serve as proof of the authenticity and integrity of transactions. Users sign transactions using their private keys, creating a unique digital signature that is tied to the transaction data and the user’s public key. Other nodes in the network can then verify the authenticity of the transaction by checking the digital signature against the public key, without revealing the private key. This mechanism ensures that only the legitimate owner of the private key can initiate transactions, providing a strong layer of security against unauthorised access and tampering.
Another crucial cryptographic mechanism in blockchain security is the use of cryptographic hash functions. A hash function is a mathematical algorithm that takes an input of arbitrary length and produces a fixed-size output, known as a hash. The properties of hash functions, such as determinism, preimage resistance, and collision resistance, make them well-suited for use in blockchain networks. In Bitcoin, hash functions are utilised in the process of mining and the creation of new blocks, with the PoW consensus algorithm requiring miners to solve complex mathematical puzzles based on the SHA-256 hash function. By design, this puzzle is computationally expensive and requires a substantial amount of computational power to solve, effectively securing the network against potential attacks by making it prohibitively expensive for malicious actors to gain control of the majority of the network’s mining power.
Additionally, hash functions play a vital role in maintaining the integrity and immutability of the blockchain. Each block in the chain contains a reference to the previous block’s hash, creating a tamper-evident link between blocks. Any alteration to a block’s contents would result in a change to its hash, breaking the link to subsequent blocks and rendering the entire chain invalid. This property of hash functions ensures the immutability of the blockchain, making it virtually impossible for attackers to alter transaction data or manipulate the network’s history.
What are the Threats that Blockchains face?
Given that the primary application for blockchain networks is somewhat akin to a digital bank, acting as a medium for transactions and the storage of user data, malicious actors are presented with an alluring monetary incentive to attack the network. Consequently, at any given moment, most major blockchains face an array of attackers looking to devise a strategy to overcome the security measures in place. These attacks are varied and diverse, tending to target the vulnerabilities inherent to blockchain architecture.
51% Attack
A 51% attack represents a significant vulnerability in the context of blockchain security, especially for networks employing PoW or PoS consensus mechanisms. This category of attack occurs when a single entity or a colluding group of entities gain control over more than 50% of the network’s mining or staking power, thereby enabling them to manipulate the blockchain’s consensus process. With this level of control, the attackers can potentially modify, reorder or censor transactions, initiate double-spend attacks, or even halt the addition of new blocks to the chain. The ramifications of a 51% attack can be severe, as it undermines the integrity, immutability, and security of the blockchain, thereby eroding trust in the network and potentially causing a decline in the value of the associated digital asset.
Understanding the mechanics of a 51% attack requires an examination of how malevolent actors exploit their majority control over the network’s resources. In a PoW system, the attacker would need to command a majority of the total hashing power, whereas, in a PoS system, they would need to control a majority of the staking power. By harnessing this power, attackers can effectively create a private, parallel version of the blockchain, often referred to as a secret or hidden chain. This parallel chain allows the attacker to execute double-spend attacks, wherein they spend the same coins on both the public and the secret chain, thereby defrauding unsuspecting recipients. Additionally, with their dominant position, the attacker can selectively censor or modify transactions in the blocks they create, undermining the fundamental principles of decentralisation and trustlessness. Ultimately, the attacker can release their secret chain to the public, and if it has more accumulated work or stake than the public chain, the network will be forced to accept it as the valid chain, solidifying the attacker’s manipulations.
Historically, there have been instances of 51% attacks on various blockchain networks, illustrating the real-world implications of this security vulnerability. One notable example occurred in 2018, when the Ethereum Classic (ETC) network suffered a 51% attack, resulting in the double-spending of more than 200,000 ETC tokens worth over $1 million at the time. Another instance took place in 2019 when Bitcoin Gold, a Bitcoin fork, experienced a 51% attack that led to the double-spending of approximately 7,000 BTG tokens, valued at around $70,000. These examples serve to highlight the inherent risks associated with 51% attacks and the need for robust security measures to protect blockchain networks.
Several countermeasures have been proposed and implemented to mitigate the risk of 51% attacks, focusing on both technical and economic aspects. On the technical side, some blockchain networks have adopted alternative consensus mechanisms, such as Delegated Proof of Stake (DPoS) or Byzantine Fault Tolerance (BFT) systems, which tend to be less susceptible to 51% attacks due to their different security assumptions and reliance on a smaller set of validators or nodes. Additionally, some projects employ a hybrid consensus model, combining PoW and PoS mechanisms to increase the complexity and resource requirements for executing a successful attack. On the economic side, strategies such as imposing significant penalties on malicious actors or implementing time-lock mechanisms to delay the spending of newly-minted coins can help deter potential attackers. Ultimately, the combination of technical and economic countermeasures aims to increase the cost and reduce the potential rewards of launching a 51% attack, thereby enhancing the overall security and resilience of blockchain networks.
Sybil Attack
A Sybil attack is a malicious attempt to undermine the trust and security of a blockchain network by creating numerous fake nodes or user identities to manipulate the consensus process. Named after the protagonist in the book “Sybil” by Flora Rheta Schreiber, who suffers from multiple personality disorder, this type of attack exploits the inherent open and permissionless nature of many decentralised systems. By masquerading as multiple distinct participants, an attacker seeks to gain undue influence over the network’s decision-making processes, which may have far-reaching consequences for the platform’s stability, security, and functionality.
The execution of a Sybil attack involves the creation of a substantial number of pseudonymous user accounts or nodes controlled by a single entity. By doing so, the attacker can disrupt the consensus mechanism by skewing the voting power in their favour or by manipulating the flow of information within the network. Depending on the specific blockchain protocol and its underlying consensus algorithm, Sybil attacks can manifest in various ways, including collusion in PoS systems, eclipse attacks in peer-to-peer networks, or spamming in distributed hash tables (DHTs). The objective of a Sybil attack is often to compromise the integrity of the network, enabling the attacker to carry out fraudulent transactions, censor specific participants, or even launch more sophisticated attacks such as double-spending or 51% attacks.
Several noteworthy examples of Sybil attacks and their potential impact on blockchain networks have been documented in both academic research and real-world scenarios. In 2015, a study by the Microsoft Research team highlighted the susceptibility of the Bitcoin network to Sybil attacks, revealing that a relatively small number of colluding nodes could effectively partition the network, thereby disrupting the propagation of transactions and blocks. Additionally, in 2016, the Ethereum network faced a large-scale Sybil attack that targeted its public nodes, forcing the developers to implement countermeasures such as increasing the minimum requirements for establishing peer connections. These incidents underscore the need for robust and adaptive security measures to protect blockchain networks from Sybil attacks and other potential threats.
In response to the risks posed by Sybil attacks, various mitigation techniques have been developed and implemented across different blockchain networks. One common approach is to introduce resource-based constraints, such as Proof of Work (PoW) or PoS, which require attackers to invest significant computational power or financial resources to create a large number of fake nodes, thereby raising the barrier of entry for launching a successful attack. Other strategies include reputation systems, which assign trust scores to nodes based on their past behaviour, and secure peer sampling protocols, which limit the attacker’s ability to manipulate network connections. While no single solution can guarantee complete immunity from Sybil attacks, the combination of these countermeasures can significantly enhance the resilience and security of blockchain networks, ensuring their continued growth and widespread adoption.
Denial of Service (DoS)
A Denial of Service (DoS) attack is a type of cyber threat that aims to disrupt the normal functioning of a blockchain network by overwhelming its resources, rendering it inaccessible to legitimate users. Similar to Sybil attacks, DoS attacks pose a significant threat to the security and stability of blockchain networks, as they exploit the network’s inherent vulnerabilities in terms of resource allocation and management. By inundating the network with an excessive volume of requests or transactions, an attacker can cause severe congestion, slow down transaction processing, and potentially lead to network downtime.
In the context of blockchain networks, DoS attacks can be executed in various ways, such as flooding the network with spam transactions, targeting specific nodes with a high volume of incoming connections, or exploiting protocol-level vulnerabilities that result in resource exhaustion. The primary objective of a DoS attack is to hinder the network’s ability to process and settle transactions, thereby undermining user confidence and the overall security of the platform.
Several high-profile cases of DoS attacks have been observed in the blockchain ecosystem, illustrating their potential impact on network security and functionality. In 2016, the Ethereum network experienced a series of DoS attacks that exploited vulnerabilities in the smart contract execution environment, leading to severe network congestion and prompting the developers to implement a series of hard forks to address the issues. Similarly, in 2017, the Bitcoin network faced a massive transaction backlog due to a sudden influx of low-fee transactions, which some experts attributed to a coordinated DoS attack aimed at disrupting the network’s operations.
To mitigate the risks associated with DoS attacks, blockchain networks employ a variety of defensive measures, such as rate-limiting, transaction prioritisation, and adaptive resource management. Rate-limiting mechanisms restrict the number of incoming connections or transactions per unit of time, preventing an attacker from flooding the network with excessive requests. Transaction prioritisation, often based on fees, ensures that high-value transactions are processed ahead of spam or low-fee transactions, reducing the impact of congestion on the network’s performance. Adaptive resource management techniques, such as dynamic block size adjustment or gas limit adjustments in Ethereum, enable the network to respond to sudden changes in transaction volume, ensuring that resources are allocated efficiently and fairly among users.
While it is challenging to completely eliminate the threat of DoS attacks, the implementation of these countermeasures can significantly enhance the resilience and security of blockchain networks. By continually refining and updating these defensive mechanisms, blockchain networks can maintain their robustness and protect users’ assets and data against potential threats.
Eclipse Attack
An Eclipse attack is a form of network-level attack that targets blockchain systems by isolating specific nodes or users from the rest of the network, effectively controlling their view of the blockchain’s state and manipulating the flow of information. This type of attack is closely related to Sybil attacks, as it often involves the creation of numerous fake nodes or user identities to establish a dominating presence within the victim’s network connections. By executing an Eclipse attack, an attacker aims to compromise the security and trust of the targeted nodes or users, potentially enabling them to carry out double-spending, censor transactions, or manipulate consensus processes.
The execution of an Eclipse attack involves strategically positioning malicious nodes within the victim’s peer-to-peer connections, effectively surrounding and isolating them from legitimate network participants. Once the attacker has gained control over the victim’s network connections, they can manipulate the flow of information, selectively relaying or withholding transactions and blocks to create a distorted view of the blockchain’s state. Depending on the specific blockchain protocol and its consensus algorithm, Eclipse attacks can have varying consequences, such as hindering block propagation, disrupting transaction validation, or enabling more sophisticated attacks like double-spending or selfish mining.
The potential impact of Eclipse attacks on blockchain networks has been demonstrated in both academic research and real-world incidents. In 2015, the same study by the Microsoft Research team that highlighted the susceptibility of the Bitcoin network to Sybil attacks also revealed that a well-orchestrated Eclipse attack could effectively partition the network, thereby disrupting the propagation of transactions and blocks. Moreover, in 2018, researchers from Boston University and MIT published a paper demonstrating the feasibility of Eclipse attacks on the Ethereum network, emphasising the need for robust security measures to protect against such threats.
To counteract the risks posed by Eclipse attacks, a range of mitigation techniques have been developed and implemented across various blockchain networks. One common approach is the use of secure peer sampling protocols, making it more difficult for an attacker to manipulate network connections by ensuring that peer selection is random and resistant to tampering. Additionally, reputation systems can be employed to assign trust scores to nodes based on their past behaviour, effectively identifying and isolating malicious nodes from the network. Furthermore, network-level countermeasures, such as limiting the number of outgoing connections per node or implementing periodic peer rotation, can help prevent the formation of dominating connections that could facilitate an Eclipse attack.
While no single solution can provide complete protection against Eclipse attacks, the combination of these countermeasures can significantly bolster the resilience and security of blockchain networks. By continually refining and updating these defensive mechanisms, blockchain networks can maintain their robustness and safeguard users’ assets and data against potential threats.
Code Exploits
Code exploit attacks constitute a significant threat to the security and integrity of blockchain networks, as they target vulnerabilities and weaknesses present in the underlying code of smart contracts or the blockchain protocols themselves. These attacks leverage flaws in the design, implementation, or execution of the code, enabling attackers to compromise the system, manipulate data, or even steal funds. As blockchain networks and smart contracts are, by nature, decentralised and immutable, once a vulnerability is exploited, it can be exceedingly challenging to rectify the situation or recover the lost assets, underscoring the critical importance of robust and secure code development practices.
To comprehend the intricacies of code exploit attacks, it is essential to delve into the methodologies employed by malicious actors to identify and exploit vulnerabilities. Typically, attackers engage in a thorough analysis of the target codebase, scrutinising its design, logic, and functionality to detect any potential weaknesses. This can include probing for vulnerabilities such as reentrancy, overflow or underflow errors, or issues with access controls or permissions. Upon discovering a vulnerability, the attacker devises a strategy to exploit the weakness, often involving the execution of carefully crafted transactions or operations that manipulate the code in unintended ways. The ultimate objective of the attacker may vary, ranging from stealing funds or sensitive data to causing disruption or reputational damage to the targeted blockchain network or smart contract.
A prime example of a code exploit attack on a layer 1 blockchain is the Bitcoin Value Overflow Incident in 2010. This event saw an attacker exploit a vulnerability in the Bitcoin protocol, enabling them to create and spend a transaction containing an output value exceeding the maximum allowed limit of 21 million Bitcoin. Consequently, this led to the creation of billions of counterfeit Bitcoin, threatening the network’s overall security and integrity. To rectify the situation, the Bitcoin community swiftly implemented a software patch, and a soft fork rolled back the affected blocks, thereby restoring the network to its pre-attack state. This incident highlights the potential implications of code exploit attacks on layer 1 blockchains and emphasises the importance of robust security measures in preventing such occurrences.
To mitigate the risk of code exploit attacks on layer 1 blockchains, various countermeasures have been proposed and implemented, focusing primarily on ensuring the security and quality of the underlying code. One essential strategy is to adopt rigorous and thorough code development practices, including the use of formal verification techniques that employ mathematical proofs to verify the correctness and security of the code. Additionally, comprehensive code audits, both internal and external, can help identify and rectify potential vulnerabilities before the code is deployed on the network.
Furthermore, implementing a multi-layered security approach that encompasses network-level, protocol-level, and cryptographic measures can enhance the overall resilience of layer 1 blockchains against code exploit attacks. For instance, leveraging advanced cryptographic techniques, such as zero-knowledge proofs and secure multi-party computation, can help bolster the security of the underlying protocols. Moreover, employing robust consensus algorithms and incentive mechanisms can ensure the proper functioning of the network, even in the presence of malicious actors.
Private and Public Blockchains: What is the Difference?
When discussing the topic of blockchain security, it is crucial to recognise the differences between private and public blockchains, as these variations in structure and governance have significant implications for their security profiles. Both private and public blockchains employ the fundamental concepts of distributed ledgers, cryptography, and consensus mechanisms, but their operational models and access controls differ substantially, leading to distinct security advantages and challenges.
Public blockchains, such as Bitcoin and Ethereum, are open networks that allow anyone to participate in the validation, transaction processing, and maintenance of the ledger. The decentralised nature of public blockchains fosters robustness against single points of failure and censorship, as the network relies on a vast array of nodes distributed globally. From a security standpoint, public blockchains benefit from their extensive and diverse network of participants, as malicious actors would have to amass a significant amount of resources and computing power to launch a successful attack. The transparent and open nature of these blockchains makes it possible for anyone to audit the network and identify potential vulnerabilities, contributing to a broader security ecosystem. However, public blockchains may also be more susceptible to certain types of attacks, such as 51% attacks or Sybil attacks, as a result of their permissionless structure.
Private blockchains, on the other hand, are permissioned networks that limit participation to a select group of entities, typically trusted organisations or individuals. These blockchains are often designed for specific use cases, such as interbank transactions, supply chain management, or enterprise applications. In contrast to public blockchains, private blockchains are characterised by centralised control and governance, as a single entity or consortium of entities is responsible for the network’s operation and maintenance. While this centralisation may be seen as a weakness in terms of resilience and censorship resistance, it can also provide certain security benefits. For instance, private blockchains can leverage their controlled environment to implement rigorous access controls, identity management protocols, and auditing processes, which can mitigate the risks associated with unauthorised access, data breaches, and insider threats.
Furthermore, private blockchains can be more agile in terms of implementing security updates and addressing vulnerabilities, as their governance structures enable faster decision-making and coordinated action. In terms of consensus mechanisms, private blockchains often use less resource-intensive algorithms, such as PBFT or Proof of Authority (PoA), which can provide faster transaction processing and lower energy consumption compared to the PoW used in many public blockchains. However, it is worth noting that the smaller size and centralised nature of private blockchains may make them more vulnerable to targeted attacks, as malicious actors could focus their efforts on compromising a limited number of critical nodes or entities.
What’s Next?
Part 2: Smart Contract Security
Within the expansive cityscape of blockchain networks, smart contracts are the bricks that make up the skyscrapers housing each decentralised application (DApp).
Smart contracts are the basis for the emergence of DApps, which are essential for the mainstream adoption of blockchain technology. Due to their autonomous and immutable nature, securing smart contracts is crucial to prevent malicious exploitation. Many smart contracts, particularly in the decentralised finance (DeFi) sector, handle or hold large sums of cryptocurrencies, presenting malicious actors with an intense monetary incentive to uncover and exploit smart contract vulnerabilities. Therefore, the threats faced by smart contract engineers are numerous making stringent security assurances a necessity.
Smart Contract Basics
Smart contracts are self-executing agreements with the terms of the contract directly written into lines of code. The code and the agreements contained therein exist across a decentralised blockchain network, ensuring their transparency, immutability, and security. The concept of smart contracts was first proposed in 1994 by computer scientist and cryptographer Nick Szabo, who recognized the potential for using computer protocols to formalise and automate the execution of contractual agreements, thereby minimising the need for intermediaries and reducing the risk of fraud, disputes, or human errors. Szabo’s idea was ahead of its time and remained dormant until the emergence of blockchain technology, which provided the perfect platform for implementing smart contracts in a decentralised, trustless environment.
The development of blockchain technology, specifically the Ethereum platform, paved the way for the practical implementation of smart contracts. Ethereum, launched in 2015 by Vitalik Buterin and a team of developers, introduced a Turing-complete programming environment called the EVM, allowing developers to create and deploy smart contracts on the platform. This breakthrough enabled the realisation of Szabo’s vision, as well as the emergence of a new generation of DApps built on top of the Ethereum blockchain. The EVM not only allowed for the deployment of smart contracts but also introduced the concept of gas, which is used to measure the computational effort required to execute a contract, making it an essential component of Ethereum’s incentive structure and a practice that would become ubiquitous across the blockchain landscape.
The primary purpose of smart contracts is to automate the execution of contractual agreements, ensuring that the terms and conditions are met and enforced without the need for intermediaries or manual intervention. Smart contracts can be utilised in a wide range of applications, such as financial services, supply chain management, digital identity, voting systems, and decentralised autonomous organisations (DAOs), among others. By leveraging the decentralised nature of the underlying blockchain technology, smart contracts provide a secure, transparent, and tamper-proof environment for executing agreements, fostering trust between parties and reducing transaction costs. The immutability of the underlying blockchain ensures that the state of a smart contract is always consistent and accurate, providing an auditable trail of all the contract’s interactions.
In terms of technical details, smart contracts are essentially pieces of code written in a programming language compatible with the underlying blockchain platform. For instance, on the Ethereum network, the most commonly used programming language for smart contracts is Solidity – a language specifically designed for writing contracts on the EVM. When a smart contract is deployed, it is compiled into bytecode, which is then stored on the blockchain as part of a unique contract address. This contract address serves as a reference point for users to interact with the smart contract, invoking its functions and triggering the execution of its code. The EVM processes these function calls, executing the smart contract’s code and updating the blockchain’s state accordingly. Notably, the execution of smart contracts is deterministic, meaning that the outcome of the contract’s functions depends solely on the input data and the current state of the blockchain.
To illustrate the workings of a smart contract, consider a simple example of an escrow contract on the Ethereum network. In this case, the smart contract would be programmed to hold and manage funds between two parties involved in a transaction, only releasing the funds when specific conditions are met. The contract could be written in Solidity and might include functions for depositing funds, checking the contract balance, and releasing funds to the recipient when the agreed-upon conditions are fulfilled. A simple escrow contract in Solidity might look like the following:
pragma solidity ^0.8.0;
contract Escrow {
address public buyer;
address payable public seller;
uint256 public amount;
bool public buyerApproval = false;
bool public sellerApproval = false;
constructor(address _buyer, address payable _seller, uint256 _amount) {
buyer = _buyer;
seller = _seller;
amount = _amount;
}
function deposit() public payable {
require(msg.sender == buyer, “Only the buyer can deposit.”);
require(msg.value == amount, “Incorrect deposit amount.”);
}
function checkBalance() public view returns (uint256) {
return address(this).balance;
}
function approve() public {
if (msg.sender == buyer) {
buyerApproval = true;
} else if (msg.sender == seller) {
sellerApproval = true;
}
if (buyerApproval && sellerApproval) {
releaseFunds();
}
}
function releaseFunds() private {
require(buyerApproval && sellerApproval, “Both parties must approve.”);
seller.transfer(address(this).balance);
}
}
When a user interacts with the escrow contract by invoking one of its functions, a transaction is created and broadcasted to the Ethereum network. This transaction includes the function’s input data, along with the sender’s digital signature and an amount of Ether to cover the gas fees associated with the contract’s execution. Once the transaction is included in a block and validated by the network’s consensus algorithm, the EVM processes the transaction, executing the smart contract’s code and updating the blockchain’s state accordingly. In the case of the escrow contract, this could involve transferring funds from the sender’s account to the contract’s address, updating the contract’s balance, or releasing funds to the recipient when the conditions are met.
The execution of smart contracts consumes computational resources, resulting in users being required to pay gas fees to incentivise validators (miners or stakers) to process and validate their transactions. The gas fees are determined by the complexity of the contract’s code and the amount of computational effort required to execute its functions. The gas system in Ethereum not only serves as an incentive mechanism for validators but also as a safeguard against malicious contracts that could potentially consume excessive resources and destabilise the network.
Common Practices for Smart Contract Security
As the digital landscape evolves and the utilisation of blockchain technology becomes increasingly widespread, the need to ensure the security and integrity of smart contracts takes centre stage. The critical role of contract testing, auditing, access control and various other security practices cannot be understated. With these practices in place, developers can work toward creating secure, dependable, and transparent blockchain systems capable of meeting the challenges of an increasingly interconnected and digital world.
Testing
Among the many practices employed to fortify smart contract security, contract testing stands as a crucial component that developers must take into consideration. At its core, contract testing is the process of rigorously validating the functionality, reliability, and security of a smart contract through a series of defined tests. These tests are designed to detect and rectify any vulnerabilities, bugs, or logic flaws that may exist within the contract’s code before it is deployed on the blockchain. Once deployed, smart contracts become immutable, meaning that any errors or security loopholes cannot be corrected without redeployment of the contract, which could potentially lead to significant financial losses or even the collapse of an entire DApp. Consequently, contract testing serves as a vital step in mitigating the risks associated with deploying smart contracts on the blockchain.
The methodology behind contract testing involves the use of multiple testing techniques, each serving a specific purpose in the evaluation of the smart contract. Unit testing, one of the most fundamental techniques, focuses on testing individual functions and methods within the smart contract in isolation. By doing so, developers can ascertain the correctness and stability of each component, allowing for the early identification and resolution of potential issues. For example, a unit test might examine whether a specific function correctly updates a user’s balance after a transaction or if it accurately calculates the rewards earned by staking tokens in a DeFi platform.
Another essential technique employed in contract testing is integration testing, which assesses how the various components of the smart contract interact with each other and with external systems. Integration tests aim to ensure that the overall functionality of the contract remains intact and coherent, even as individual components are combined and connected to external resources such as oracles or other smart contracts. An integration test might, for instance, verify that a decentralised exchange (DEX) smart contract correctly interacts with a price oracle to obtain real-time asset prices, subsequently ensuring that the platform executes trades at the appropriate market rates.
In addition to these testing techniques, developers may also utilise formal verification methods to mathematically prove the correctness of their smart contracts. While complex and often resource-intensive, formal verification can provide a higher degree of confidence in the contract’s security and reliability by ensuring that its code adheres to a formal specification. This level of scrutiny is particularly beneficial for high-value contracts or those that handle critical infrastructure within a blockchain ecosystem.
The importance of contract testing is exemplified by numerous high-profile incidents in the blockchain space, such as the infamous DAO hack in 2016, which resulted in the loss of approximately $60 million worth of Ether. The attack was facilitated by a vulnerability in the DAO smart contract, allowing an attacker to exploit the contract’s logic and syphon off funds. Had the smart contract undergone rigorous contract testing, the vulnerability might have been detected and rectified before deployment, preventing the catastrophic event and its subsequent consequences.
Code Reviews and Audits
As the adoption of blockchain technology and smart contracts continues to gain momentum, ensuring the security and reliability of these digital agreements becomes increasingly crucial. While practices such as contract testing serve to enhance the integrity of smart contracts, another indispensable measure in the quest for secure and dependable code is the implementation of code reviews and audits. These systematic examinations of smart contract code are conducted by experienced developers or specialised security teams, with the primary objective of identifying and addressing potential vulnerabilities, errors, or inefficiencies that may have been overlooked during the development process. By meticulously scrutinising the code, auditors aim to uncover any weak points that could be exploited by malicious actors or lead to unintended consequences, thereby safeguarding users’ assets and preserving the credibility of the underlying blockchain ecosystem.
Code reviews and audits can be executed in various forms, ranging from informal peer reviews to comprehensive, professional audits conducted by specialised firms. In the case of informal peer reviews, members of the development team or the broader community assess each other’s code, providing constructive feedback and recommendations for improvements. This collaborative approach fosters knowledge-sharing and encourages best practices, ultimately enhancing the overall quality of the smart contract. On the other end of the spectrum, professional audits carried out by reputable security firms offer a more rigorous and structured assessment of the code, leveraging their expertise and experience to detect subtle vulnerabilities that may elude less seasoned developers. A professional audit typically entails a thorough examination of the smart contract’s functionality, compliance with industry standards, and adherence to established security practices, culminating in the production of a detailed audit report that documents the findings and provides actionable recommendations for remediation.
The necessity of code reviews and audits is underscored by numerous high-profile incidents that have plagued the blockchain space, exposing vulnerabilities in smart contracts and resulting in substantial financial losses. For instance, the 2017 Parity wallet hack, which led to the loss of over $150 million worth of Ether, can be traced back to a critical flaw in the wallet’s smart contract code. This vulnerability allowed an attacker to exploit the contract’s multi-signature functionality and gain unauthorised access to users’ funds. Had the smart contract undergone a rigorous audit, the vulnerability might have been identified and addressed prior to deployment, averting the disastrous event and the ramifications it brought on.
In light of such incidents, code reviews and audits have become an indispensable aspect of smart contract security, serving to reinforce users’ trust and confidence in the technology. By subjecting their code to meticulous scrutiny, developers can proactively identify and rectify vulnerabilities, reducing the risk of exploitation and enhancing the overall security of the blockchain ecosystem. As the prevalence of smart contracts continues to grow, the importance of robust code reviews and audits will only become more pronounced, highlighting the need for rigorous security practices and a steadfast commitment to ensuring the integrity and resilience of these digital agreements. Consequently, the implementation of thorough code reviews and audits will remain an essential element in the ongoing pursuit of secure, dependable, and transparent blockchain systems that can withstand the challenges of an increasingly interconnected and digitalised world.
Access Control and Upgradeability
Smart contract security is an indispensable component of blockchain ecosystems, and among the various practices employed to enhance the safety and reliability of these digital agreements are access control and contract upgradeability. Access control refers to the implementation of mechanisms within smart contracts that restrict unauthorised users from executing specific functions or modifying sensitive data. By establishing a clear hierarchy of permissions, access control measures ensure that only authorised parties can interact with specific aspects of the smart contract, thereby mitigating the risk of unauthorised tampering or exploitation. Contract upgradeability, on the other hand, pertains to the ability to modify or enhance a smart contract’s code after its initial deployment, enabling developers to address unforeseen vulnerabilities, implement new features, or adapt to changes in the broader blockchain ecosystem.
Access control mechanisms are often implemented through the use of access modifiers and role-based access control (RBAC) systems. Access modifiers are employed to restrict the visibility and usage of certain functions within a smart contract, limiting their accessibility to specific users or user groups. These modifiers can be applied to functions or state variables, effectively creating a permission system that defines which parties are authorised to interact with certain aspects of the contract. RBAC systems take this concept a step further by assigning specific roles to users, each with its own set of permissions and restrictions. By mapping roles to individual users or user groups, developers can granularly control access to sensitive functions or data, ensuring that only authorised parties can execute specific actions or modify critical aspects of the smart contract. Implementing access control measures is of paramount importance in safeguarding users’ assets and preserving the overall security and integrity of the blockchain ecosystem.
Contract upgradeability is another critical aspect of smart contract security, as it enables developers to address vulnerabilities, enhance functionality, or adapt to changing circumstances in the blockchain space. Traditional smart contracts are immutable by design, meaning that their code cannot be altered once deployed. While this characteristic offers certain benefits in terms of trust and transparency, it also poses significant challenges when it comes to addressing bugs or vulnerabilities that may emerge over time. To tackle this issue, developers can employ various techniques to make smart contracts upgradeable, such as using proxy contracts or employing a modular design. Proxy contracts act as intermediaries between users and the underlying smart contract logic, allowing developers to swap out the underlying implementation without disrupting the contract’s interface or state. Modular designs, on the other hand, involve separating the smart contract’s logic into distinct components that can be individually upgraded or replaced, providing a flexible and adaptive architecture that can evolve alongside the blockchain ecosystem.
The importance of access control and contract upgradeability is exemplified by numerous incidents in the blockchain space, where lapses in security or the inability to address vulnerabilities have resulted in significant financial losses or reputational damage. For instance, the aforementioned Parity wallet hack could have been mitigated through stringent access control measures that prevented unauthorised users from exploiting the smart contract’s multi-signature functionality. Similarly, the aforementioned DAO hack of 2016, might have been averted had the smart contract been designed with upgradeability in mind, allowing the developers to promptly address the critical vulnerability that was exploited by the attacker.
How Does Smart Contract Security Differ Between Different Applications?
Smart contract security is a multifaceted domain that requires tailored approaches depending on the specific application in which the contracts are employed. The unique challenges and requirements associated with each application necessitate distinct security measures and best practices to ensure the safety and reliability of smart contracts. In this context, understanding the nuances and distinctions of smart contract security across various use cases is crucial for ensuring the robustness and integrity of these digital agreements.
Layer 2s
Layer 2 networks, designed to enhance the scalability and performance of the underlying blockchains, demand a heightened focus on data availability and fraud proofs. Data availability ensures that the main chain can access the data required to validate transactions on the secondary layer. Various techniques can be implemented to address data availability issues, such as erasure coding, which enables efficient data recovery, and data availability sampling, which allows nodes to randomly sample and verify small portions of data.
Fraud proofs play a vital role in maintaining the integrity of layer 2 networks. To secure smart contracts in layer 2 networks, developers must design robust fraud proof mechanisms, including interactive dispute resolution protocols and cryptographic commitments. These mechanisms help participants monitor and challenge invalid transactions, providing an additional layer of security for layer 2 chains.
DeFi
DeFi platforms, which rely on smart contracts to facilitate financial products and services, require a particular emphasis on the accuracy and integrity of pricing oracles. Developers can adopt various strategies to mitigate risks associated with DeFi smart contracts, such as using time-weighted average prices (TWAP) oracles, providing a more accurate representation of an asset’s value over time. Implementing circuit breakers to halt trading activities during periods of extreme price volatility can also help safeguard against potential attacks.
Additionally, developers should employ multiple oracles or decentralised oracle networks to increase the reliability and security of data feeds. By implementing mechanisms to validate and cross-check data from different sources, they can further reduce the potential for price manipulation attacks and enhance overall DeFi smart contract security.
Governance
In the realm of governance, smart contracts manage critical decision-making processes and resource distribution for DAOs or blockchain-based voting systems. Secure voting mechanisms, such as quadratic voting or token-weighted voting, can help mitigate the risks of voting manipulation and ensure the integrity of governance processes. These mechanisms consider the participants’ token holdings or assign voting power based on a mathematical function to prevent undue influence.
Developers can also counter potential Sybil attacks and bribery schemes in governance smart contracts by implementing decentralised identifiers (DIDs) for secure identity management and using cryptographic techniques, such as zero-knowledge proofs, to preserve the anonymity and privacy of voting participants.
Bridges
Blockchain bridges, which enable interoperability and cross-chain functionality, require specific security approaches to facilitate the transfer of assets and data between different blockchain networks. One essential aspect of bridge security is ensuring the accuracy and integrity of data transmitted between networks. Developers can achieve this by implementing secure multi-party computation (SMPC) protocols, which allow multiple parties to collaboratively perform computations while preserving the privacy of their individual inputs.
Another critical aspect of bridge security is the validation and verification of transactions across different chains. Developers can employ mechanisms such as cryptographic proofs, including Merkle proofs and zero-knowledge proofs, to validate transactions and ensure data consistency across chains. By combining these approaches, developers can enhance the security and robustness of smart contracts used in blockchain bridges, fostering trust in cross-chain communication.
Other
In addition to the specific security considerations outlined above, various other applications of smart contracts may require tailored security measures. For instance, non-fungible tokens (NFTs) leverage smart contracts to manage the ownership and transfer of unique digital assets. In this context, developers should focus on ensuring the authenticity and provenance of the assets, implementing mechanisms such as digital signatures and on-chain metadata to verify the origins and history of the NFTs.
In the case of supply chain management, smart contracts can be used to track and verify the movement of goods throughout the entire supply chain. Security concerns in this area include data integrity and privacy. Developers can utilise cryptographic techniques, such as secure hashing algorithms and encryption, to protect sensitive data and maintain the confidentiality of business information. Additionally, they can employ consensus algorithms that cater to the specific requirements of supply chain networks, ensuring the reliability and trustworthiness of the recorded data.
With respect to digital identity management, smart contracts can be employed to create decentralised identity systems that offer greater control and privacy for users. Security considerations in this domain involve user privacy, data protection, and access control. Developers can implement techniques including zero-knowledge proofs and secure multi-party computation to preserve user privacy while allowing for the verification of identity claims. Furthermore, they can design access control mechanisms based on cryptographic primitives, such as attribute-based encryption, to ensure secure and fine-grained access to sensitive identity data.
What Are the Threats Smart Contracts Face?
As the use of blockchain technology expands, smart contracts have emerged as a powerful tool for automating complex transactions and enforcing contractual agreements in a decentralised and trustless manner. However, with increased adoption comes a heightened focus on the security of these digital contracts. A variety of threats can undermine the integrity, reliability, and trustworthiness of smart contracts, making it essential for developers, users, and organisations to be aware of potential vulnerabilities and adopt best practices to protect their platforms and applications. By analysing the different types of threats and their associated risks, it becomes possible to implement effective countermeasures that contribute to the resilience and robustness of smart contracts within the blockchain ecosystem.
Reentrancy Vulnerabilities
The 2016 DAO hack, resulting from a reentrancy vulnerability, is one of the most notorious examples of smart contract vulnerability. The attacker exploited a recursive call pattern that allowed them to repeatedly drain Ether from the contract before the balance was updated. This incident served as a wake-up call for the industry, highlighting the importance of stringent security measures and best practices in smart contract development. Reentrancy vulnerabilities arise when a smart contract allows external calls to other contracts or addresses during the execution of a function, enabling the called contract to reenter the original function before it has completed execution. This can lead to unexpected outcomes, including multiple withdrawals or unauthorised actions if not properly guarded against.
To mitigate the risk of reentrancy attacks, developers can implement checks-effects-interactions patterns, utilise mutexes or reentrancy guards, and carefully analyse the potential for recursive calls in their contract code. In addition, thorough testing and code reviews can help identify potential reentrancy vulnerabilities and ensure the implementation of robust security measures.
Integer Overflow and Underflow Vulnerabilities
Integer overflow and underflow vulnerabilities are another prevalent threat to smart contract security. These occur when arithmetic operations result in values that exceed the maximum or minimum storage capacity of a particular data type, causing the value to “wrap around” or “roll over” to an unintended value. For instance, the infamous batchOverflow vulnerability in the ERC-20 token standard allowed attackers to generate an arbitrarily large number of tokens by exploiting integer overflow. This led to a rapid devaluation of the affected tokens and disrupted the operation of several cryptocurrency exchanges.
To address this type of vulnerability, developers can employ SafeMath libraries, which include built-in overflow and underflow checks for arithmetic operations, or implement custom overflow and underflow protection mechanisms in their contract code. Additionally, rigorous testing, peer reviews, and adherence to secure coding practices can further minimise the risks associated with integer overflow and underflow vulnerabilities.
Logic Flaws
Logic flaws in smart contracts can expose them to various risks. These flaws can be the result of coding errors, incorrect assumptions, or oversights in the contract’s design or implementation. For example, a poorly implemented access control mechanism could allow unauthorised users to execute privileged functions or manipulate critical data, leading to unintended outcomes, including the loss of funds or the compromise of the entire contract.
To prevent logic flaws, developers should adhere to established coding standards, conduct thorough testing and code reviews, and leverage formal verification techniques when possible to ensure the correctness of their smart contract logic. Additionally, employing modular design principles and seeking feedback from the developer community can contribute to more robust and secure smart contract implementations.
Race Conditions and Transaction Ordering Attacks
In the context of smart contracts, race conditions occur when multiple transactions or contract functions are executed concurrently, and their outcomes depend on the order in which they are processed. If not properly accounted for, race conditions can create opportunities for malicious actors to exploit timing discrepancies and manipulate the contract’s state or behaviour. Developers can minimise the risk of race conditions by employing atomic operations, implementing time locks or commit-reveal schemes, and meticulously analysing transaction ordering and timing assumptions in their contract code.
External Dependencies and Oracle Manipulation Attacks
Smart contracts can also be vulnerable to external dependencies, such as oracle manipulation attacks. Oracle manipulation occurs when an attacker exploits the reliance of a smart contract on an external data source, or oracle, to feed it false or manipulated information, ultimately causing the contract to execute unintended actions or transfers. A notable example of an oracle manipulation attack was the bZx hack in 2020, where the attacker manipulated the price of an asset on a decentralised exchange, enabling them to profit from the discrepancy between the manipulated and true asset values.
To mitigate the risk of oracle manipulation attacks, developers should meticulously evaluate the trustworthiness of the oracles and external data sources they rely on. Utilising multiple oracles or decentralised oracle networks, such as Chainlink, can help increase the reliability and security of data feeds used in smart contracts. Implementing mechanisms to validate and cross-check data from different sources can also reduce the potential for oracle manipulation.
Additionally, developers should consider employing robust fallback mechanisms in case of oracle failure or data discrepancies, as well as implementing monitoring systems to detect suspicious activities or potential attacks. Regularly auditing the security of the oracles and third-party dependencies, and remaining up-to-date with the latest security best practices and developments in the blockchain ecosystem, can further contribute to the resilience of smart contracts against external dependency-related vulnerabilities.
Other
Aside from the primary vulnerabilities discussed above, there are additional attack vectors that can pose a threat to smart contract security. These attacks may exploit more subtle vulnerabilities or target aspects of the blockchain ecosystem that are not directly related to the smart contract code. However, understanding and mitigating these risks is essential to ensuring comprehensive security for smart contract platforms and applications.
Front-running is a type of attack that exploits the transparency of blockchain transactions and the race for block space among competing transactions. In a front-running attack, a malicious actor monitors the transaction pool (also known as the mempool) for pending transactions that involve a profitable opportunity, such as a large trade on a DEX. The attacker then submits their own transaction with a higher gas price, incentivising network participants (validators/miners) to prioritise it over the original transaction. By executing their transaction first, the attacker can capitalise on the market impact of the original transaction and profit at the expense of the victim. Developers can mitigate front-running risks by implementing measures such as commit-reveal schemes, cryptographic techniques like zero-knowledge proofs, or leveraging layer 2 solutions that offer transaction confidentiality.
Sybil attacks are another potential threat to smart contract security, particularly in the context of decentralised systems that rely on consensus mechanisms or voting processes. In a Sybil attack, an attacker creates multiple fake identities or nodes to gain disproportionate influence over the network, potentially allowing them to manipulate the consensus or voting outcomes. While Sybil attacks do not directly exploit smart contract code, they can compromise the integrity of the underlying blockchain and impact the security and reliability of smart contracts built on top of it. Developers can defend against Sybil attacks by utilising robust consensus algorithms, such as Proof of Stake (PoS) or Proof of Authority (PoA), making it more difficult and costly for an attacker to control a significant portion of the network.
Social engineering attacks pose a more indirect threat to smart contract security but can be just as damaging if successful. These attacks target the human element of the blockchain ecosystem, exploiting psychological manipulation and deception to gain unauthorised access to sensitive information, private keys, or privileged functions. While social engineering attacks do not exploit technical vulnerabilities in the smart contract code, they can still result in significant losses or compromise of the contract if the attacker gains access to critical information or resources. To mitigate the risk of social engineering attacks, developers should prioritise security awareness training, enforce strict access controls and multi-factor authentication, and establish clear policies for handling sensitive information and responding to potential security incidents.
What’s Next?
Part 3: The Future of Blockchain and Smart Contract Security
Future Threats
As the world of blockchain technology continues to advance at a rapid pace, it becomes imperative to anticipate and prepare for potential threats that may emerge in the future. With innovations such as quantum computing on the horizon, new challenges arise that could potentially disrupt the current security mechanisms in place. This relentless progression in adversarial techniques will differentiate those protocols that can keep up with the rapidly evolving pace of technological development from those that fall behind, further underscoring the importance of remaining proactive and adaptable in the face of an ever-changing technological landscape.
Quantum Computing
The development of quantum computing has the potential to revolutionise many industries by offering significant increases in computational power. This emerging technology relies on quantum bits, or qubits, which can exist in multiple states simultaneously, enabling quantum computers to perform complex calculations at unprecedented speeds. While this technological advancement promises many benefits, it also poses a significant threat to the security of all systems vulnerable to brute force attacks and other computationally intensive hacking practices. A brute force attack is a common hacking method that uses continuous trial and error until the correct value is inputted, commonly used for cracking passwords. While traditional computers don’t possess the requisite power to carry out brute force attacks efficiently, quantum computers are orders of magnitude more powerful than even the most powerful supercomputers.
Current cryptographic algorithms employed by blockchains, such as the elliptic curve digital signature algorithm (ECDSA) and secure hash algorithm (SHA), which are widely used to secure blockchain networks, rely on the computational infeasibility of solving certain mathematical problems for their security. However, quantum computers have the potential to solve these problems efficiently, rendering traditional cryptographic algorithms vulnerable to attacks. This could compromise the integrity of blockchain networks, allowing attackers to decrypt sensitive data, manipulate transactions, or even double-spend assets. To address this looming threat, researchers and developers are exploring post-quantum cryptography, aiming to create cryptographic algorithms that can withstand the computational capabilities of quantum computers and ensure the continued security of blockchain networks.
The Emergence of More Advanced Hacking Techniques
As technology continues to advance, so do the methods and techniques employed by malicious actors to exploit vulnerabilities within blockchain systems. The emergence of more sophisticated hacking tools and techniques presents a significant challenge for blockchain security. For instance, machine learning algorithms can be used by attackers to identify patterns and weaknesses in blockchain networks more effectively than humans, while novel attack vectors such as side-channel attacks or consensus algorithm manipulations can pose new threats to the integrity of blockchain systems.
In order to effectively combat these emerging threats, it is crucial for developers, security researchers, and the broader blockchain community to stay informed about new hacking techniques and continuously update their security practices. This includes implementing robust security measures, conducting regular audits, and fostering a culture of security awareness and education among users. The blockchain community will have to evolve their security practices alongside the hackers or risk their visage of indomitable security being cracked. By staying vigilant and adaptive, the blockchain ecosystem can effectively mitigate the risks posed by advanced hacking techniques and maintain the security and integrity of its networks.
Scalability, Centralisation and Interoperability Challenges
As the adoption of blockchain technology grows, so do the challenges associated with scaling and interoperability. Scalability refers to the ability of a blockchain network to handle an increasing number of users and transactions without sacrificing performance. Many existing blockchain networks, such as Bitcoin and Ethereum, have faced issues with transaction throughput and network congestion, which can impact security by making the network more susceptible to attacks, such as denial-of-service (DoS) or spam attacks. While many networks have begun implementing measures to improve scalability, these are not without their own challenges, namely centralisation.
Centralisation arises as a result of the concentration of power or control within certain components of a blockchain network. In the context of scaling, centralisation may arise as a result of concentration of mining/validation power in certain nodes or layer 2 scaling solutions, although scaling is but one of the numerous possible causes. This centralisation can create single points of failure that can be targeted by attackers, posing a significant risk to the security and integrity of the network. Addressing these challenges requires a combination of innovative solutions, such as sharding, layer 2 scaling solutions, and decentralised consensus mechanisms, as well as a commitment to maintaining decentralisation while achieving the desired scalability.
Interoperability, on the other hand, refers to the ability of different blockchain networks to communicate and interact with one another. As various blockchain networks seek to establish connections and collaborate with one another the challenges of cross chain communication can result in potential security vulnerabilities. Specifically, the risk of cross-chain attacks and the spread of vulnerabilities between networks may increase. This highlights the need for robust security measures and standards to ensure the secure exchange of information and assets between different blockchain networks.
Other
Evolving smart contract vulnerabilities: As smart contracts become more complex and feature-rich, the potential for new vulnerabilities and attack vectors increases. This is particularly true in the DeFi space where the complexity of financial instruments is reflected in the smart contracts that support them. Staying ahead of these emerging threats requires ongoing research, development, and collaboration within the blockchain community. Regular audits, code reviews, and the implementation of secure development practices can help to minimise the risk of smart contract vulnerabilities and ensure the continued security of blockchain networks.
Privacy Concerns: Privacy is an important aspect of security within the blockchain ecosystem. Many blockchain networks, such as Bitcoin and Ethereum, offer a degree of pseudonymity, but the public nature of their transaction ledgers can still enable the de-anonymization of users or the tracking of transaction patterns. This can pose risks to user privacy and security, making it important for developers to explore and implement privacy-enhancing technologies, such as zero-knowledge proofs, confidential transactions, or privacy-focused blockchain networks, in order to protect users’ identities and sensitive information.
Social engineering: Social engineering attacks involve the manipulation of individuals to gain unauthorised access to sensitive information or systems. These attacks can pose a significant risk to blockchain security, as even the most robust security measures can be rendered ineffective if users are tricked into revealing their private keys or other sensitive information. To combat social engineering attacks, it is crucial for users and developers especially to be educated about the risks and best practices to protect their digital assets, such as using hardware wallets, enabling multi-factor authentication, and being cautious when interacting with unknown parties.
What’s Next?
Part 4: A Novel Method of Securing Blockchains: Consensus Agnostic Immutability
The Problem
Consensus between participants lies at the core of each and every layer 1 blockchain that has been built thus far. These systems, supported by consensus mechanisms and algorithms are indispensable for the facilitation of cooperation and coordination between nodes, which in turn is necessary to ensure the immutability of the blockchain itself. However, relying on consensus still introduces inherent vulnerabilities, namely 51% attacks, Sybil attacks, DoS attacks and other manipulations that pose challenges to the majority remaining in a replicated, agreed upon state of the blockchain. Therefore, what is needed is a system whereby an innumerable number of bad actors can be present in a network without affecting the security of the honest participants, ergo ensuring the integrity of the ledger regardless of any potential threat.
Blockchain networks, especially those that are smaller and have less distributed hashing power, are particularly susceptible to the threat of 51% and Sybil attacks. This is due to the relative ease with which a malicious actor can amass enough resources (computational power or capital) to control the majority of the network’s nodes. While larger networks like Bitcoin and Ethereum, due to their expansive and globally distributed miner/validator base, pose a substantially high cost and complexity for executing such an attack, smaller and less decentralised networks do not offer the degree of security.
The spectre of a 51% and Sybil attack presents a significant hurdle for the growth and security of smaller public blockchains. These networks often struggle to amass enough nodes to secure their network adequately, making them attractive targets for bad actors. In the absence of ample network security, these blockchains can suffer substantial losses due to double-spending attacks, undermining their credibility and user trust. Moreover, the fear of such attacks can deter potential users and investors, creating a barrier to widespread adoption. The threat of 51% attacks and other manipulations of the network thus emphasises the importance of having a sufficiently decentralised and secure network. It is a critical factor for smaller blockchains to consider and address if they aspire to grow, gain users’ trust, and ensure the long-term stability of their platform.
Over the years, several instances of 51% attacks have been documented. One of the most notable ones happened with Ethereum Classic (ETC), a smaller, forked version of the Ethereum network, in January 2019. The attack led to a loss of over $1 million in double-spent ETC tokens. The attacker took advantage of the low hashing power of the Ethereum Classic network to reorganise the blockchain and double-spend tokens. This attack left a considerable dent in Ethereum Classic’s reputation, and its value dropped significantly following the incident. The network has since taken steps to improve its security, but the event still serves as a significant example of the potential threats that smaller blockchains face.
Another infamous example was the attack on Verge (XVG) in 2018. The attacker exploited a bug in the Verge code to mine new blocks quickly, effectively controlling the network for a few hours. During this period, they were able to mine around 250,000 XVG (around $1.75 million at the time) before the network could respond. The incident significantly affected the credibility of Verge’s blockchain and served as a stark reminder of the potential risks associated with cryptocurrencies, particularly those with smaller node networks. These incidents, among others, underscore the need for robust security measures and sufficient decentralisation in blockchain networks.
The Solution: Consensus Agnostic Immutability
Given the risks of attacks on consensus between nodes, the blockchain landscape is in need of a mechanism that can maintain the state of an immutable ledger accurately between a network of participants without requiring consensus. Although numerous present day systems maintain an immutable ledger without consensus, these systems are typically centralised, relying on a primary node or otherwise to inform network participants regarding the correct state. Thus, these systems are impractical for the blockchain landscape considering decentralisation and trustlessness compose the underlying principles of the technology.
Therefore, what is needed is a system that can maintain an immutable ledger between a decentralised network of participants interacting without trust assumptions. In order to ensure the security of the ledger, this system must be able to operate with an innumerable number of bad actors present in the network without affecting the security of the honest participants, ergo ensuring the integrity of the ledger regardless of any potential consensus-related threat. This model would require the creation of an immutable ledger across a distributed network without relying on consensus between network participants. Such a model would enable the creation of a blockchain that is invulnerable to 51% attacks, Sybil attacks or any other manipulation of the ledger by a malicious actor.
This system, henceforth referred to as Consensus Agnostic Immutability (CAI), functions via a mechanism of state replication between honest nodes. Transactions are executed utilising simple checks, similarly to traditional blockchains such as Bitcoin or Ethereum. Each transaction is broadcast to the entire network so that each node can participate in the process of block validation (this is a common practice occurring in most blockchains). A node in the network is then chosen to propose the next block using an established method such as PoW or PoS. Before broadcasting the proposed block, the chosen node first broadcasts its Merkle root of the blockchain itself. Each node in the network then compares it to its own Merkle root. If the state is valid, then the node accepts the proposer as a valid network participant and proceeds to validate and finalise the proposed block.
However, if the broadcast state is deemed invalid by the receiving node (deviating from their own maintained state), then the receiving node automatically rejects the proposer as a valid network participant. Said node would then proceed to coordinate with other network participants that also disagreed regarding the state of the network to select a new block proposer. Consequently, each node that accepted the block produced by the invalid node would reflect an invalid state on future rounds. Subsequently, a network fork is created between the nodes that accepted the proposed block and those that rejected the proposed block. Therefore, moving forward the nodes in each fork can then only propose blocks to the nodes in their fork that maintain the same state. Hence, the nodes in the invalid fork are unable to interact with the honest nodes maintaining the valid state of the network.
The Infrastructure
While CAI-based networks excel in terms of decentralisation and security, the indispensability of ubiquitous state replication to the operation of the network imposes inherent scalability constraints. Therefore, the implementation of scalability mechanisms is indispensable as they would drastically increase the processing and storage capacity of the network. Furthermore, users require a reliable means to differentiate between valid and invalid forks, without which the network becomes unusable. Without these core properties of scalability and usability, the enhanced security and decentralisation of a CAI-based network would mean very little.
Sharded/Modular Network
This can be achieved via the implementation of modularity, within the CAI blockchain itself and also within the larger protocol. One way to achieve modularity within the blockchain is via a sharding mechanism. Sharding works by partitioning the network into smaller, manageable parts, or ‘shards’, each capable of processing transactions and smart contracts in parallel. This approach could significantly increase the network’s transaction capacity and reduce latency, thereby improving overall performance.
However, maintaining security and preserving the network’s ability to identify valid forks in a sharded architecture presents its own challenges. One way to address these issues could be to implement a system of shard verification that uses a mechanism analogous to CAI’s block validation process. In this proposed sharding mechanism, each shard would function as a mini-CAI network, maintaining its own ledger and following the CAI model of state replication between honest nodes within the shard. Just as in the original CAI model, if a node within a shard attempts to propose an invalid block, the other nodes within the shard would reject the proposed block, leading to the creation of an invalid fork within that shard.
Now, to maintain security between shards and ensure that all shards can identify the valid fork of each shard, a ‘cross-shard’ validation mechanism could be introduced. This mechanism could involve the use of ‘checkpoint’ blocks, which are blocks that contain a summary of the state of each shard, proposed at regular intervals. Each shard would then broadcast its checkpoint block to all other shards, allowing them to compare the state of their shard with the checkpoint block’s state. If there is agreement, the shard accepts the checkpoint block; if there is disagreement, the shard rejects the checkpoint block, creating a network fork on the level of the shards.
To further secure cross-shard transactions and ensure the recognition of valid forks, a group of oracles, dubbed ‘cross-shard oracles’, could be established. These cross-shard oracles would continually monitor a particular shard, calculating the current state and differentiating between valid and invalid forks. Each shard could then utilise the data broadcast by cross-shard oracles to identify the valid checkpoint block. In order to mitigate the risk of cross-shard oracle fraud, participation would be heavily incentivised and layers of redundancy added such as the rotation of cross-shard oracles between shards and the implementation of an inter-shard reputation mechanism, whereby each cross-shard oracle, whenever validating the same shard, rates its peer regarding their reliability.
In addition to implementing intra-blockchain modularity via sharding, the addition of inter-blockchain modularity may allow CAI-based networks to achieve previously unseen levels of scalability. Inter-blockchain modularity, more commonly referred to as blockchain modularity, involves the separation of the core components of blockchain operation, that being data availability, chain consensus, transaction settlement and transaction execution, into separate networks. While the consensus layer would be unnecessary in a CAI based network due to the existence of a verifiably canonically valid fork, the separation of the responsibilities of data availability and storage, and transaction execution and settlement into separate networks would enable a large increase in the scalability and processing power of a CAI based network.
Data Structures and Decentralised Communication
State Storage
Efficient state storage presents a crucial and challenging aspect of CAI networks. An effective solution to this persistent issue can be found in the application of a Merkle Patricia Trie (MPT). While Verkle tries may be a better option if implemented correctly, MPTs are tried and tested, having served as the backbone of several blockchain implementations, including Ethereum. The MPT is a modified version of a Merkle trie that carries the capacity to store all account states. This trie’s structure offers a compact, secure, and verifiable method of representing the state of accounts within the network. Its value lies in its root hash – a unique identifier stored in the block that enables nodes to verify the entirety of the state against this reference point. This feature enhances the integrity and reliability of the CAI network, allowing it to efficiently manage a large number of account states while minimising the risk of errors or inconsistencies.
To further optimise state storage, techniques such as pruning can be employed. Pruning refers to the selective removal of historical data from the blockchain’s storage that is no longer necessary for its ongoing operation. Once transactions are validated and blocks are added to the blockchain, certain types of data, such as transaction details and intermediary states, can be safely discarded without compromising the chain’s integrity. The pruning process can help maintain a lean and efficient blockchain, reducing storage requirements and improving the speed and performance of the network.
Data Transmission
To minimise the size of transactions and optimise the data transmission across the CAI network, techniques such as transaction compression and aggregation can be implemented. Transaction compression utilises more efficient data structures or encoding mechanisms to represent transaction data, thereby reducing the overall data size. This compression can result in significant space savings, allowing more transactions to be included in a single block and improving the overall throughput of the network.
Transaction aggregation offers another layer of optimisation. It involves the combination of multiple transactions into a single one. Advanced cryptographic techniques such as ZK-SNARKs (Zero-Knowledge Succinct Non-Interactive Argument of Knowledge) or ZK-STARKs (Zero-Knowledge Scalable Transparent Arguments of Knowledge) allow multiple transactions to be aggregated into a single proof. This proof serves as a condensed representation of multiple transactions, reducing the amount of data that needs to be stored on-chain and thereby improving the scalability of the network.
The application of off-chain transactions can also significantly reduce the size of messages circulated within the network. Layer 2 solutions offer an opportunity to batch multiple transactions off-chain, with only the final state of these transactions being written on-chain. This approach further optimises data transmission by reducing the amount of data required to be written to and read from the blockchain.
P2P Communication
A cornerstone of effective operation within a CAI network is the capacity for P2P communication. A Gossip protocol could facilitate this communication need. In such a protocol, nodes share their information randomly with their peers, who then propagate it further, ensuring that all nodes eventually receive all necessary information. This includes proposed blocks, executed transactions, and state data. The Gossip protocol forms a decentralised communication network that is robust, scalable, and able to withstand failures or attacks.
To further optimise network dissemination, enhancements can be made to the Gossip protocol. For instance, mechanisms could prioritise the distribution of more critical or newer information, ensuring the most relevant data is disseminated quickly throughout the network. Nodes could also be incentivised to act as “super nodes” and handle a larger share of the network’s communication. These super nodes can help speed up data propagation and make the network more robust and reliable.
Implementing a system such as libp2p, a modular network stack, could provide additional benefits. Libp2p allows the network to switch between different transports, use multiple addresses, and dynamically discover peers. This adaptability can improve the network’s resilience, make it more adaptable to different environments and network conditions, and enhance the efficiency of communication between nodes.
Block Explorer
In the context of a CAI network, the potential creation of invalid network forks, despite the existence of robust disincentives, presents a significant challenge. Ensuring the capacity to distinguish between valid and invalid nodes is a paramount requirement for the network’s integrity and user trust. The absence of an effective differentiation mechanism can lead to users inadvertently connecting to and transacting with invalid nodes. Even though negative externalities resulting from such connections would not influence the valid fork, they could precipitate significant user frustration. To address this fundamental concern, we propose a robust and efficient solution that enables users to accurately identify valid nodes through an oracle-connected block explorer.
The primary element of this solution is a specially configured oracle node. Unlike conventional nodes, the oracle node continually processes transactions occurring within the network but cannot propose new blocks. This specific functionality ensures that the oracle node’s operations remain purely observational, thereby largely eliminating any potential for conflicting interests that might otherwise arise from the proposal of new blocks. By eschewing block proposal capabilities, the oracle node is capable of accurately calculating the current state of the network and differentiating between valid and invalid forks, an invaluable resource for all network participants.
Building upon the capabilities of the oracle node, a block explorer is connected and acts as an interface for users, ranging from individual transactors to DApps, smart contracts, and other network entities. The block explorer harnesses the oracle’s discerning capability to provide users with up-to-date, accurate information regarding the current valid network fork. With a user-friendly interface that reflects the network’s true state, users are better equipped to make informed decisions when interacting with the network, reducing the risk of inadvertent transactions on invalid forks.
A vital aspect of the oracle node’s design is its contribution to maintaining the network’s trustless nature. By making the oracle node’s codebase open source, any participant within the network can set up and operate their own oracle. This strategy enhances transparency and maintains the ethos of decentralisation that underpins the network. By allowing individual verification of the network’s state, we remove trust assumptions that might arise when relying on a single oracle node. Each participant can thus independently affirm the validity of the network state, fostering an environment of trust and cooperation within the CAI network.
This system architecture also plays a pivotal role in minimising the potential for centralisation and manipulation. As each user can operate their own oracle, the risk of a single point of failure or control is significantly mitigated. In the event one oracle is compromised, others will continue to provide accurate information about the network’s state, maintaining the overall integrity and resilience of the CAI network. While this approach has been discussed primarily in the context of a monolithic CAI network, the same could be applied within a modular network. This decentralised approach aligns with the network’s fundamental principles, while also providing a robust solution to potential adversarial actions.
Mechanism Design Safeguarding CAI
In the context of game theory, CAI provides strong incentives for participants to engage honestly and maintain the valid state of the network. Nodes that maintain the legitimate state and interact in good faith with the rest of the network are rewarded with the continued ability to participate in the network. They can interact with other honest nodes, process transactions, and contribute to the blockchain’s overall security and health. Moreover, they gain reputational advantage, trust, and the potential for rewards associated with block validation and transaction fees.
CAI effectively disincentivises dishonest behaviour through the imposition of stringent punitive measures. Any deviation from the valid state automatically triggers the rejection of proposed blocks by nodes maintaining this state. Therefore, when a node attempts to manipulate the network state, it is automatically excluded from effective participation, isolating it from the honest network participants. This means that any stakes they had in the network are essentially nullified, and they lose the ability to process transactions and partake in the benefits of the network. Furthermore, their influence on the network diminishes as they are confined to an isolated, invalid fork.
This essentially results in a form of self-ostracization, acting as a potent deterrent for potential bad actors. Their subsequent transactions go unrecorded on the valid fork and are therefore completely reversible for any users that accidentally transact using an invalid node. Given that the blockchain operates on principles of transparency and decentralisation, this loss of status and functionality forms a strong disincentive for dishonest participation, thereby creating a highly secure network environment.
Given that nodes storing an invalid state are nullified from the perspective of nodes storing the valid state, their stake in the network is similarly nullified. This nullified stake becomes irretrievable and is burned from the perspective of the valid fork. Thus, fraudulent behaviour can actually benefit the valid participants by introducing a deflationary pressure on the native cryptocurrency.
CAI also anticipates the potential creation of invalid network forks, despite the severe disincentives, and proposes measures to protect users from accidentally transacting with these invalid nodes. This is accomplished through aforementioned oracle nodes that continually monitor and report on the current valid network state, thereby ensuring that users are transacting on the valid fork. These oracle nodes, made open source, would enable any user to differentiate between valid and invalid network forks. This system not only guards against the consequences of potentially interacting with dishonest nodes, but further incentivizes honest participation. Knowing that invalid transactions and dishonest nodes will be identified and isolated gives users confidence in the network’s robustness and security.
Under a CAI system, the benefits of honest participation are accentuated, and the consequences of dishonest actions are exacerbated. A malicious actor attempting to infiltrate the valid network is faced with a daunting prospect of achieving little more than censorship and transaction reordering, while the risk of complete isolation and nullification of their stake is imminent. Even attempts at censorship and reordering would be mitigated via the node-to-node transaction querying mechanism, which would largely bypass transactions from inefficient nodes, thereby disincentivising any potential manipulative behaviour. All these factors combine to create a highly secure and resilient network, where the game theory incentives align in favour of honest participation, thereby effectively rendering the blockchain immune to attacks.
Applications of CAI
The implementation of a CAI system can provide substantial improvements for both Layer 1 and Layer 2 blockchain solutions. Layer 1 blockchains, such as Bitcoin and Ethereum, provide the foundational infrastructure for decentralised applications. They are responsible for transaction validation, block production, and maintaining the overall security of the network. However, as these networks have grown in size and popularity, they have faced significant challenges in scalability and speed. The CAI model provides a pathway to improve these aspects without compromising the decentralised and secure nature of these networks.
For layer 1 blockchains, a CAI system would bring forth an advanced, resilient structure that does not rely on traditional consensus for maintaining the accuracy of the ledger. By operating under a mechanism that relies on state replication between honest nodes, smaller and less established layer 1 blockchains could significantly improve their resistance to common security threats such as 51% attacks and Sybil attacks. This resilience and security are critical to their growth, as it would allow them to gain the trust of users and compete more effectively with larger, more established networks. Furthermore, the application of a CAI system would enhance the overall robustness of layer 1 blockchains, enabling them to support a higher number of decentralised applications and handle increased transaction volume without compromising on security or decentralisation.
Moreover, the application of CAI mechanisms in layer 2 solutions, such as rollups, is just as significant. Layer 2 solutions are built atop layer 1 blockchains to improve scalability and speed. They process transactions off-chain, batch them, and then submit them to the Layer 1 network. Despite their potential to address the scalability issues of layer 1 blockchains, layer 2 solutions often rely on a single, centralised sequencers to order and batch transactions, potentially becoming points of failure.
A CAI system, integrated with layer 2 solutions, could provide a decentralised method for selecting and rotating these sequencers, thus reducing the risk associated with a single point of control. The incorporation of a CAI system would offer a layer of security and decentralisation to these layer 2 solutions, allowing them to handle a larger volume of transactions with greater speed and efficiency. By facilitating secure and efficient transaction processing, layer 2 solutions implementing CAI could significantly enhance the user experience, driving adoption and fostering a more inclusive, decentralised, and efficient blockchain ecosystem.
FAQs
What is blockchain security?
Blockchain security refers to the measures and mechanisms in place to ensure the integrity, transparency, and immutability of data stored on a blockchain network, protecting it from vulnerabilities, attacks, and malicious exploits.
How does decentralization enhance blockchain security?
Decentralization distributes the control and maintenance of the network across multiple nodes rather than a single central authority. This inherently discourages manipulation and fraud, reduces the chances of a single point of failure, and minimizes the potential for malicious attacks.
What is the Byzantine Generals Problem in blockchain security?
The Byzantine Generals Problem is a theoretical challenge in distributed computing that illustrates the difficulties of achieving consensus in a network where some participants might act maliciously or transmit false information. In the blockchain context, consensus mechanisms like Proof of Work (PoW) and Proof of Stake (PoS) are designed to address this problem, ensuring network agreement even in the presence of malicious actors.
How does Proof of Work (PoW) contribute to blockchain security?
PoW involves network nodes, or miners, competing to solve complex cryptographic puzzles that require significant computational power. Successfully solving the puzzle allows a miner to add a new block to the blockchain. This competitive and resource-intensive nature ensures nodes remain honest, making attacks highly improbable and economically unfeasible.
What is the difference between Proof of Work (PoW) and Proof of Stake (PoS) in terms of security?
While both PoW and PoS are consensus mechanisms to validate transactions and add new blocks, PoW relies on computational power to solve cryptographic puzzles, whereas PoS emphasizes the role of economic incentives, tying the likelihood of validating blocks to the amount of tokens a node holds or “stakes” as collateral.
How does blockchain settlement ensure security?
Transaction settlement refers to the process of finalizing, verifying, and securely adding transactions to the distributed ledger. It ensures that transactions are valid before finalizing them into blocks, safeguarding the network from fraud and malicious activities and providing users with confidence in the completion of their transactions.
What are the security implications of public vs. private blockchains?
Public blockchains, like Bitcoin and Ethereum, are open to everyone, allowing any participant to engage in transactions and contribute to the network’s security. In contrast, private blockchains restrict access to a selected group, often controlled by a central authority, tailored to cater to specific organizational needs. While public blockchains benefit from widespread decentralization, private blockchains might have enhanced control mechanisms but might lack the same level of decentralization.
How do layer 2 solutions impact blockchain security?
Layer 2 solutions are built atop the base layer of existing blockchain networks to enhance scalability, throughput, and latency. They process transactions off-chain and settle the results on the base layer, reducing the strain on the main network. While they can improve performance, their security implications depend on their specific design and integration with the base layer.
What role do nodes play in blockchain security?
Nodes, which are computers or servers participating in the network, maintain a copy of the entire blockchain, constantly validating and relaying transaction data. Full nodes store the complete blockchain history and execute all transaction validations, acting as the backbone of the blockchain network and preserving its security and decentralization.
How do smart contracts factor into blockchain security?
Smart contracts are programmable scripts running on the blockchain that automate and facilitate the execution of predefined conditions and agreements without intermediaries. While they can enhance the functionality and automation of blockchain networks, they also introduce potential vulnerabilities if not properly coded or audited, making their security crucial to the overall integrity of the network.
DISCLAIMER
Zerocap Pty Ltd carries out regulated and unregulated activities.
Spot crypto-asset services and products offered by Zerocap are not regulated by ASIC. Zerocap Pty Ltd is registered with AUSTRAC as a DCE (digital currency exchange) service provider (DCE100635539-001).
Regulated services and products include structured products (derivatives) and funds (managed investment schemes) are available to Wholesale Clients only as per Sections 761GA and 708(10) of the Corporations Act 2001 (Cth) (Sophisticated/Wholesale Client). To serve these products, Zerocap Pty Ltd is a Corporate Authorised Representative (CAR: 001289130) of AFSL 340799
All material in this website is intended for illustrative purposes and general information only. It does not constitute financial advice nor does it take into account your investment objectives, financial situation or particular needs. You should consider the information in light of your objectives, financial situation and needs before making any decision about whether to acquire or dispose of any digital asset. Investments in digital assets can be risky and you may lose your investment. Past performance is no indication of future performance.
Like this article? Share
Latest Insights
Interview with Ausbiz: How Trump’s Potential Presidency Could Shape the Crypto Market
Read more in a recent interview with Jon de Wet, CIO of Zerocap, on Ausbiz TV. 23 July 2024: The crypto market has always been
Weekly Crypto Market Wrap, 22nd July 2024
Download the PDF Zerocap is a market-leading digital asset firm, providing trading, liquidity and custody to forward-thinking institutions and investors globally. To learn more, contact
What are Crypto OTC Desks and Why Should I Use One?
Cryptocurrencies have gained massive popularity over the past decade, attracting individual and institutional investors, leading to the emergence of various trading platforms and services, including
Receive Our Insights
Subscribe to receive our publications in newsletter format — the best way to stay informed about crypto asset market trends and topics.